
effect of normobaric
hypoxia on sound localisation
Operators of modern military
aircraft must acquire and process large amounts of information to perform their
tasks effectively. Traditionally, aircraft designers have relied heavily on
visual displays as a means of presenting information. Audio displays also have
considerable potential for conveying information within this environment.
Auditory signals can capture attention and provide information without the need
for continual visual monitoring. Well-designed audio displays could complement
or replace visual displays and reduce the visual workload currently experienced
by aircrew.
Substantial progress has
recently been made toward the development of three-dimensional (3D) audio
displays that generate sound that is heard in 3D space. These
displays work by passing auditory signals through location-specific digital
filters that modify these signals as the head and ears would. It is envisaged
that 3D audio displays will inform future aircrew of such things as the nature
and location of threats to their aircraft. Several recent studies
have shown that these displays could assist aircrew in their search for small
visual targets such as other aircraft.
How reliably 3D audio displays
will function within the aviation environment will depend in part on the extent
to which auditory perception is affected by hypoxia. Previous studies have shown
that hypoxia impairs various aspects of visual perception and general cognitive
functioning. Of particular relevance with respect to 3D audio
displays, however, is hypoxia's potential effect on sound localisation.
Hypoxia's effect on sound
localisation has been examined in only two studies. Rosenberg and
Pollard measured the localisation accuracy of subjects involved in separate
mountaineering expeditions to the Andes, Himalayas and Alps. Sounds to be
localised consisted of short bursts of broadband noise presented over
headphones. These sounds had been recorded at the eardrums of an acoustic
manikin while broadband noise was presented from a speaker at different azimuthal locations in the manikin’s interaural horizontal plane. They therefore
contained the sound location cues imposed on the noise by the manikin’s head and
pinnae. Rosenberg and Pollard observed a significant deterioration in sound
localisation accuracy for subjects involved in each expedition at altitudes
ranging from 1455 to 5360 m. In a subsequent laboratory study, Rosenberg et al. measured the localisation accuracy of subjects at sea level and simulated
altitudes of 1524, 2438, 3657 and 5486 m in a hypobaric chamber. As before,
sounds to be localised consisted of short bursts of broadband noise pre-recorded
through the ears of an acoustic manikin. The accuracy with which subjects
localised these sounds was observed by Rosenberg et al. to be significantly
lower at the two higher altitudes. At both of these altitudes many subjects
displayed localisation errors of a directionally systematic nature but the
direction toward which they mislocalised was inconsistent across subjects.
It is possible that the altitude
at which hypoxia begins to affect sound localisation is lower than the studies
by Rosenberg and Pollard and Rosenberg et al. suggest. Two types of
localisation error are commonly distinguished. Errors of the first, and most
common, type are relatively small and occur where the location of a sound source
is more-or-less correctly perceived. Errors of the second type are known as
front-back confusions and occur where the angle of a sound source with respect
to the median plane is correctly perceived but the source is judged to be in the
incorrect hemisphere (front or back). Rosenberg et al. (19) reported an average
localisation error of about 24O at sea level and a front-back
confusion rate that averaged 42% and did not differ with altitude. Rosenberg and
Pollard reported an average localisation error of 22-26o at sea level
and did not report front-back confusion rates. These values are extremely high
in relation to those normally measured in studies in which sounds are presented
from a speaker in a free field. The poor performance at sea level in Rosenberg and Pollard's and Rosenberg et al.'s studies may have produced a floor
effect that made it difficult to demonstrate performance reduction at altitudes
above sea level.
As described above, the sounds
to be localised in the studies by Rosenberg and Pollard and Rosenberg et
al. were recordings that had been physically filtered by the head and pinnae of an acoustic manikin. The cues used by humans to localise sound are
known to be highly individualistic and it is likely that subjects in these
two studies localised poorly because the cues provided by the manikin were not
well matched to those normally available to them. It is also possible that the
localisation performance of these subjects was hampered by a paucity of
effective high-frequency content in the sounds to be localised. While the
hearing of each subject in the study by Rosenberg et al., for example, was
reported to be "acceptable" or "good" in accordance with RAF standards, hearing
was tested for frequencies up to 6 kHz only. As the ages of subjects in that
study ranged from 19 up to 43 years, it is likely that some had reduced
sensitivity for frequencies above that value. Frequencies above 6 kHz are known
to provide important cues to sound location.
Sound-source locations in
Rosenberg and Pollard's (18) and Rosenberg et al.'s (19) studies were restricted
to the 0° elevation plane. The elevation of a sound source is
determined using cues that differ from those used to determine its azimuth (see
13 for review). It is possible, therefore, that hypoxia has different effects on
elevation and azimuth judgements. As it is envisaged that 3D audio displays will
provide both elevation and azimuth information, the effect of hypoxia on the
localisation of sound presented from a broader range of elevations needs to be
examined.
In this study, hypoxia’s effect
on sound localisation was examined for sound presented from a free-field source
using subjects whose ability to accurately localise this stimulus at sea level
had been established. This ensured that any reduction in performance resulting
from hypoxia would be readily apparent. The range of locations for which
hypoxia's effect on sound localisation has been examined was extended to include
locations outside the 0° elevation plane.
Methods
Subjects
Four healthy, right-handed
volunteers, one female and three male, ranging in age from 25 to 40 years
participated in this study. Informed consent was obtained from each. Hearing was
assessed by measuring absolute thresholds for 1, 2, 4, 8, 10, 12, 14 and 16 kHz
pure tones using procedures described in detail by Watson et al. For each
subject all thresholds were lower than the relevant age-specific norm.
All subjects were allowed to
practice sound localisation during several training sessions prior to data
collection. During this period each subject's performance stabilised at a level
that demonstrated his/her proficiency at the sound localisation task.
Design
Localisation performance was
measured at each of four simulated altitudes (0, 1200, 2400 and 3700 m above sea
level). Each subject participated in four experimental sessions during each of
which a different altitude was tested. The order in which altitudes were tested
was counterbalanced across the four subjects using a Latin square.
Induction of hypoxia
Hypoxia was induced at sea level
and ambient atmospheric pressure by having subjects breathe an appropriate gas
mixture through an aviator's oxygen mask. Mixtures having oxygen concentrations
of 21.0, 18.1, 15.6 and 13.3 % were used to simulate altitudes of 0, 1200, 2400
and 3700 m, respectively. All were blended from bottled air, oxygen and nitrogen
no more than 30 minutes before the session in which they were used. These
mixtures were stored in 100 litre Douglas bags until needed. Testing in each
session did not begin until the subject had breathed the relevant gas mixture
for at least 15 minutes and his/her blood oxygen saturation level, which was
monitored throughout via non-invasive pulse oximetry, had stabilised at an
altitude-appropriate level. The saturation levels at which individual subjects
stabilised for each altitude are shown in Table 1. An interval of at least one
hour separated all pairs of consecutive sessions. No subject experienced hypoxia
for more than two sessions on any day.
Altitude (m)
|
Subject 1
|
Subject 2
|
Subject 3
|
Subject 4
|
0
|
97
|
100
|
96
|
98
|
1200
|
95
|
96
|
94
|
96
|
2400
|
95
|
93
|
91
|
92
|
3700
|
88
|
|
80
|
83
|
Table 1. Blood oxygen saturation levels (%) for
individual subjects for each altitude. Unfortunately, either no value was
recorded for subject 2 at 3700 m or the recorded value was misplaced.
|
Localisation Procedure
The subject was seated in a
sound-attenuated anechoic chamber at the centre of a 1-m radius hoop on which a
loudspeaker was mounted. Background noise levels within
this chamber were less than 10 dB SPL in all 1/3-octave bands with centre
frequencies from 0.5 to 16.0 kHz. Loudspeaker movement was driven by
programmable stepping motors that could position the loudspeaker anywhere from 0
to 359.9o azimuth and —50 to +80o elevation with .1o
resolution. A convention of measuring azimuth in the clockwise direction and
describing elevation below the interaural horizontal plane as negative was
followed. The subject's view of the hoop and loudspeaker was obscured by a cloth
sphere supported by thin fibreglass rods. The cloth from which this sphere was
constructed was acoustically transparent. The inside of this sphere was dimly
lit to allow visual orientation. Subjects wore a headband on which a laser
pointer and a magnetic tracker receiver were
mounted.
At the beginning of each trial
the subject fixated on an LED at 0o azimuth and elevation. When
ready, he/she pressed a hand-held button. An acoustic stimulus was then
presented from the loudspeaker, provided the subject's head was stationary (its
azimuth, elevation and roll did not vary by more than 0.2o over three
successive readings of the head tracker made at 20 ms intervals). This stimulus
consisted of a fresh, 328-ms burst of broadband (.05-20 kHz) noise incorporating
20-ms cosine-shaped rise and fall times. Its level was set at 60 dB (A-weighted)
using a microphone and sound-level meter positioned at the centre of the hoop. The subject was instructed to keep
his/her head stationary during stimulus presentation. Following stimulus
presentation, the subject directed the laser pointer beam at the precise point
on the surface of the cloth sphere from which he/she perceived the stimulus to
come. The location and orientation of the laser pointer was measured by the
magnetic tracker so that the point where the beam intersected the sphere could
be calculated geometrically. An LED attached to the centre of the loudspeaker
was illuminated and the subject then directed the laser pointer beam at the LED.
The angle between the two vectors extending from the centre of hoop to the
perceived location of the stimulus source and the loudspeaker LED was defined as
the localisation error.
The stimulus location for each
trial was chosen following a pseudorandom procedure that ensured a more-or-less
even spread within each session. The part-sphere from —47.6 to +80o
elevation was divided into 42 sectors of similar shape and area. One of these
sectors was chosen without replacement on each trial and a location within it
was chosen randomly. The loudspeaker was moved to the target location in two
steps to reduce the likelihood of subjects discerning the location from the
duration of movement. During the first step the loudspeaker was moved to a
randomly chosen location at least 30o away from the previous and
subsequent target locations in both azimuth and elevation. During the second
step it was moved to the target location.
Results
For each subject the average
localisation error was calculated for each simulated altitude. The average
localisation error at each altitude averaged across all four subjects is shown
in Figure 1. Errors for trials on which front-back confusions were made are
included in these averages and have not been modified in any way. The average
localisation error at sea level (0 m) was a little less than 14o,
reflecting the good localisation performance of these subjects. Average
localisation errors at all higher altitudes were a little smaller and ranged
from 12.6 to 12.9o. The error bars shown in this figure represent one
standard error of the average localisation error. Their small magnitude
indicates that average localisation errors varied little across subjects.
Figure 1.
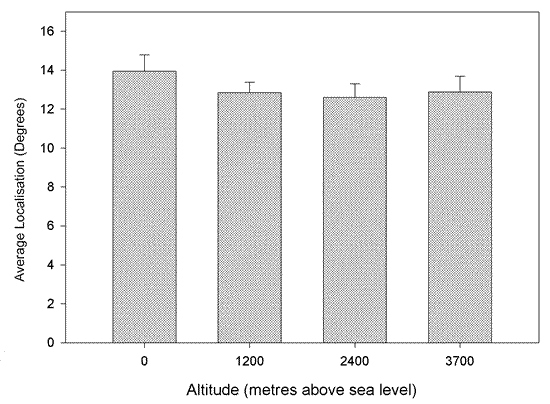
Average localisation error at each
altitude averaged across all four subjects.
An analysis of variance
incorporating a Greenhouse-Geisser correction indicated that the effect of
altitude on average localisation error was not significant (F(1.36,4.08)=.54,
p=.505). The power of this analysis was calculated following procedures outlined
by Keppel (9) and found to be sufficient („.8) to detect an effect of 5 to 6o.
While it is possible that a smaller effect was present but failed to reach significance
due to lack of power, the magnitude of the altitude effect, as measured by an
estimate of omega-squared (9), was found to be negligible (ω2=-.095).
Discussion
The results of this study
indicate that hypoxia induced by exposure to simulated altitudes as high as 3700
m has no discernible effect on sound localisation. This finding is in contrast
with those of Rosenberg and Pollard and Rosenberg et al. who observed
a significant deterioration of sound localisation ability in subjects exposed to
altitudes as low as 1455 m.
It is unlikely that the null
result obtained in this study resulted from our use of a relatively small number
of subjects. Analysis of our data revealed the presence of sufficient power to
detect a 5 to 6o effect, which is of similar size to, or smaller
than, the effects observed by Rosenberg and Pollard and Rosenberg et al.. In addition, the only trend evident in our data, which was in the
direction of improved localisation performance at higher altitude, was
shown to be of negligible magnitude.
It was suggested above that
Rosenberg and Pollard and Rosenberg et al. might have overestimated
the altitude at which hypoxia begins to have a deleterious effect on sound
localisation. This suggestion was made because of the poor sea-level performance
of their subjects and the possible existence of a floor effect that made it
difficult to demonstrate performance reduction at higher altitudes. It was
argued that our use of a free-field localisation task would avoid this
possibility and provide a particularly sensitive measure of localisation
accuracy. As reported above, the average localisation error at sea level for our
subjects was 14o. This, as expected, is considerably smaller than the
localisation errors of subjects in the studies by Rosenberg and Pollard and
Rosenberg et al. and is more in keeping with those observed in previous
free-field localisation studies. The good sea-level performance
of our subjects provided a baseline against which a reduction in performance at
higher altitudes would have been readily apparent. Despite this, however, we did
not observe any performance reduction at altitudes up to 3700 m.
It is not clear why Rosenberg
and Pollard and Rosenberg et al.observed a deterioration in sound
localisation performance at altitudes below 3700 m and we did not. It has
already been noted that Rosenberg and Pollard and Rosenberg et al.
presented stimuli via a non-individualised 3D audio display while stimuli in our
study were presented via a free-field source. Another obvious difference between
these studies concerns the ambient pressure conditions under which hypoxia was
induced. Rosenberg and Pollard and Rosenberg et al. induced hypoxia
under hypobaric conditions by exposing their subjects to high altitude
environments or low pressure in a hypobaric chamber. We induced hypoxia under
normobaric conditions by reducing the oxygen concentration in the gas
mixture inspired by our subjects at sea level. Changes in ambient pressure
create pressure differences across the eardrum that can reduce auditory
sensitivity and distort sound localisation cues unless pressure is equalised by
opening the Eustachian tube. It is possible that subjects in Rosenberg et al.'s study localised inaccurately at altitudes above sea level because the
pressure across their eardrums was not completely equalised. This is unlikely
with respect to subjects in Rosenberg and Pollard's study, however, as they
had ample time to achieve equalisation during the gradual physical ascent to
each higher altitude. The effect of ambient pressure change in the absence of
hypoxia could be examined by exposing subjects to low pressure in a hypobaric
chamber while allowing them to breath a gas mixture with an appropriately
enhanced oxygen concentration.
The results of this study are
encouraging with respect to the application of 3D audio displays within the
aviation environment. Many operators within that environment are routinely
exposed to low levels of hypoxia and all are at risk of exposure to much higher
levels. In view of the fact that severe levels of hypoxia have been found to
induce significant reductions in cochlear sensitivity in non-human subjects, it seems reasonable to assume that localisation will be compromised
under conditions of extreme hypoxia. As hypoxia levels normally experienced by
operators of modern aircraft are below the maximum in this study, however, our
results indicate that localisation performance in that environment will not
normally be affected by hypoxia.
|