
building an aircraft -
the raw materials
By Chris Heintz
Aircraft structures are basically unidirectional. This means that one
dimension, the length, is much larger than the others - width or height.
For example, the span of the wing and tail spars is much longer than their
width and depth; the ribs have a much larger chord length than height
and/or width; a whole wing has a span that is larger than its chords or
thickness; and the fuselage is much longer than it is wide or high. Even a
propeller has a diameter much larger than its blade width and thickness,
etc.... For this simple reason, a designer chooses to use unidirectional
material when designing for an efficient strength to weight structure.
Unidirectional materials are basically composed of thin, relatively
flexible, long fibres which are very strong in tension (like a thread, a
rope, a stranded steel wire cable, etc.)
An aircraft structure is also very close to a symmetrical structure. That
means the up and down loads are almost equal to each other. The tail loads
may be down or up depending on the pilot raising or dipping the nose of
the aircraft by pulling or pushing the pitch control; the rudder may be
deflected to the right as well as to the left (side loads on the
fuselage). The gusts hitting the wing may be positive or negative, giving
the up or down loads which the occupant experiences by being pushed down
in the seat ... or hanging in the belt.
Because of these factors, the designer has to use a structural material
that can withstand both tension and compression. Unidirectional fibres may
be excellent in tension, but due to their small cross section, they have
very little inertia (we will explain inertia another time) and cannot take
much compression. They will escape the load by bucking away. As in the
illustration, you cannot load a string, or wire, or chain in compression.
In order to make thin fibres strong in compression, they are "glued
together" with some kind of an "embedding". In this way we can take
advantage of their tension strength and are no longer penalized by their
individual compression weakness because, as a whole, they become
compression resistant as they help each other to not buckle away. The
embedding is usually a lighter, softer "resin" holding the fibres together
and enabling them to take the required compression loads. This is a very
good structural material.
wood
Historically, wood has been used as the first unidirectional structural
raw material. Nature, in her wisdom, has provided a beautiful
unidirectional material by making certain trees grow in certain
conditions: They have to be tall and straight and their wood must be
strong and light. The cross section of a tree trunk shows the "annual
rings" (a ring per year so that we can
"count" the tree's age). The dark bands (late wood) contain many fibres,
whereas the light bands (early wood) contain much more "resin". Thus the
wider the dark bands, the stronger and heavier the wood. If the dark bands
are very narrow and the light bands quite wide, the wood is light but not
very strong. To get the most efficient strength to weight ratio for wood
we need a definite numbers of bands per inch (see ANC No. 18,1951). In
fact, what we want is a good balance of "early" and "late" wood, or in
other words, very special growing conditions, i.e., the geographic
altitude where the tree's growth varies with the latitude and local
climatic conditions. Although this is a very interesting subject we will
not go further into such details except to mention that it is nature who
supplies us with a very efficient material from its plant kingdom.
Remember that contrary to the strictly mineral world hopelessly subject to
gravity pulling everything down, the plant has a force within itself which
makes it grow against gravity, upwards. If we could use those life forces
in our machines we could lift off without the help of an engine...
Aviation still has a lot to discover....
Another subject we will not deal with this month is the testing of wood.
There are a few simple tests (humidity, dynamics, resilience) but it seems
that nobody knows them anymore.
Some of our aircraft structures are two-dimensional (length and width are
large with respect to thickness). Plywood is often used for such
structures. Several thin boards (foils) are glued together so that the
fibres of the various layers cross over at different angles (usually 90
degrees today years back you could get them at 30 and 45 degrees as well).
Plywood makes excellent "shear webs" if the designer knows how to use
plywood efficiently. (We will learn the basis of stress analysis sometime
later.)
To close this discussion on wood, let us plainly state the fact that our
present day bureaucratic civilization uses so much paper that we are
depleting the planet of trees without replanting them correctly. Today
good aircraft wood is very hard to come by. Instead of using one good
board for our spars, we have to use laminations because large pieces of
wood are practically unavailable, and we no longer can trust the wood
quality; we have to use many laminations so that the "average" has a
reasonable chance to give us the required strength without too much
penalty from a weight standpoint. From an availability point of view, we
simply need a substitute for what nature has supplied us with until now.
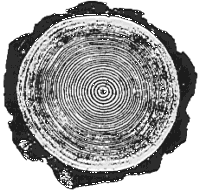
aluminium alloys
So, since wood may not be as available as it was before, we look at
another material which is strong, light and easily available at a
reasonable price (there's no point in discussing Titanium - it's simply
too expensive). Aluminium alloys are certainly one answer. We will discuss
the properties of those alloys which are used in light plane construction
in more detail later. For the time being we will look at aluminium as a
construction material.
Extruded Aluminium Alloys: Due to the manufacturing process for aluminium
we get a unidirectional material quite a bit stronger in the lengthwise
direction than across. And even better, it is not only strong in tension
but also in compression. Comparing extrusions to wood, the tension and
compression characteristics are practically the same for aluminium alloys
so that the linear stress analysis applies. Wood, on the other hand, has a
tensile strength about twice as great as its compression strength;
accordingly, special stress analysis methods must be used and a good
understanding of wood under stress is essential if stress concentrations
are to be avoided!
Aluminium alloys, in thin sheets (.016 to .125 of an inch) provide an
excellent two dimensional material used extensively as shear webs - with
or without stiffeners - and also as tension/compression members when
suitably formed (bent).
It is worthwhile to remember that aluminium is an artificial metal. There
is no aluminium ore in nature. Aluminium is manufactured by applying
electric power to bauxite (aluminium oxide) to obtain the metal, which is
then mixed with various strength-giving additives. (In a later article, we
will see which additives are used, and why and how we can increase
aluminium's strength by cold work hardening or by tempering.) All the
commonly used aluminium alloys are available from the shelf of dealers.
When requested with the purchase, you can obtain a "mill test report" that
guarantees the chemical and physical properties as tested to accepted
specifications. (MIL standards, QQA250 XYZ).
As a rule of thumb, aluminium is three times heavier, but also three times
stronger than wood. Steel is again three times heavier and stronger than
aluminium.
steel
The next material to be considered for aircraft structure will thus be
steel, which has the same weight-to-strength ratio of wood or aluminium.
Apart from mild steel which is used for brackets needing little strength,
we are mainly using a chrome-molybdenum alloy called AISI 413ON or 4140. (AISI
.1025 is no longer available.)
The common raw materials available are tubes and sheet metal. Steel, due
to its high density, is not used as shear webs like aluminium sheets or
plywood. Where we would need, say a .100" plywood, a .032 inch aluminium
sheet would be required, but only a .010 steel sheet would be required,
which is just too thin to handle with any hope of a nice finish. That is
why a steel fuselage uses tubes also as diagonals to carry the shear in
compression or tension and the whole structure is then covered with fabric
(light weight) to give it the required aerodynamic shape or desired look.
It must be noted that this method involves two techniques: steel work and
fabric covering.
The advantage of 4130N steel structure is that it can readily be welded
together. This applies especially in North America where the welder does
not have to be "approved" as he has to be in Europe and Australia. This
difference in regulations, historically, has to do with the "pioneer
spirit" and explains why welded steel fuselages are so common here and
practically nowhere else.
We will be discussing tubes and welded steel structures in more detail
later and go now to "artificial wood" or composite structures.
composite materials
The designer of composite aircraft simply uses fibres in the desired
direction exactly where and in the amount required. The fibres are
embedded in resin to hold them in place and provide the required support
against buckling. Instead of plywood or sheet metal which allows single
curvature only, the composite designer uses cloth where the fibres are
laid in two directions .(the woven thread and weft) also embedded in
resin. This has the advantage of freedom of shape in double curvature as
required by optimum aerodynamic shapes and for very appealing look
(importance of aesthetics).
Today's fibres (glass, nylon, Kevlar, carbon, whiskers or single crystal
fibres of various chemical composition) are very strong, thus the
structure becomes very light. The drawback is very little stiffness. The
structure needs stiffening which is achieved either by the usual discreet
stiffeners, -or more elegantly with a sandwich structure: two layers of
thin uni- or bi-directional fibres are held apart by a lightweight core
(foam or "honeycomb"). This allows the designer to achieve the required
inertia or stiffness.
From an engineering standpoint, this method is very attractive and
supported by many authorities because it allows new developments which are
required in case of war. (The U.S. having no titanium or chromium needs to
develop practical alternatives.) But this method also has its drawbacks
for homebuilding: A mould is needed, and very strict quality control is a
must for the right amount of fibres and resin and for good adhesion
between both to prevent too "dry" or "wet" a structure. Also the curing of
the resin is quite sensitive to temperature, humidity and pressure.
Finally, the resins are active chemicals which will not only produce the
well known allergies but also the chemicals that attack our body
(especially the eyes and lungs) and they have the unfortunate property of
being cumulatively damaging and the result (in particular deterioration of
the eye) shows up only years after initial contact.
Another disadvantage of the resins is their limited shelf life, i.e., if
the resin is not used within the specified time lapse after manufacturing,
the results may be unsatisfactory and unsafe.
Finally unless the moulds are very well designed, manufactured and
maintained, the outside of the structure needs an often underestimated
amount of "elbow grease" to provide the desired finish. Also a lot of care
must be exercised as sanding down too much will result in a weaker
structure. Historically, composites had their peak a couple of years ago.
Today it is known (and proven by all those homebuilder "workshops") that
only specialists can come up with a reliable and perfect structure and
even the specialists take a chance on their own health.
summary
Nature provides a raw material beautifully suited to aircraft structures.
Unfortunately we are exploiting nature and today it is hard to find
supplies of wood and plywood of the required sizes and quality.
Aluminium alloys in extruded and laminated form are an attractive
alternative especially as they are easy to supply with guaranteed
properties.
Steel tubing continues to be very popular in North America as welding does
not seem to create any problems as feared in other parts of the world. A
tubular structure is fabric covered.
Composites can be looked at as "artificial wood" from a structural
standpoint. Like everything artificial, it can be better than the natural
product but the manufacturer needs to incorporate in the manufacturing
process the wisdom provided by nature and/or the quality provided by other
raw material's manufacturers (aluminium, chrome moly steel). This is in
addition to an expensive mould, and the hazards to our own health (and our
family's health when building in the basement).
Designing a new
aircraft, or redesigning (modifying) an existing design, should
be done by the amateur builder only with the help of a reputable
light aircraft designer. The following situations are to be
avoided: 1) too heavy a structure and, 2) not a strong enough
airframe. Anyone who has been around amateur builders and
designers long enough has seen them tapping on their wings or
fuselage and saying, "That's strong enough", but is it really?
At low speed and high load factors, say a 75 degree bank and a
speed just over 2.5 times the stall speed, the aerodynamic load
is inclined some 20 to 30 degrees forward. Will this wing which
may even have been "sand bag tested" in the "normal" load
condition stand it? Or will the wing, which has been improved
from a NACA 23012 profile by adding the "STOL" nose cuff to
improve its original abrupt stall characteristics (because not
correctly twisted), stand up to the new torsional loads due to a
four-fold increase in the twisting moment coefficient (cm from
-.008 to -18)?
Such loads are usually associated with an increase in cruise
speed, say from 130 mph to 150 mph, by increasing the original
design horsepower from 100 bhp to 150 bhp. This will further
increase the torsion load on the wing by a factor of (150/130)2.
Will your "new" wing stand those loads? If you are not sure, you
better ask somebody who really knows.
We will discuss the above formulas within the course of this
series, but for this month's column, we will stick strictly to a
comparison of materials. The values themselves should not be
used as design data.
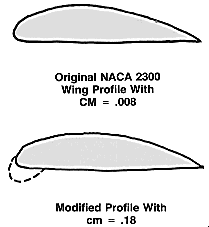
The is our Table which gives typical values for a variety of raw
materials.
Column 1 lists the standard materials which are easily
available at a reasonable cost. As this column is not intended
to be an "academic lecture," we will not discuss "fantastic"
materials because we cannot afford them anyway. We want to
acquire a simple, good understanding of practical solutions and
practical materials.
Some of the materials that fall along the borderline between
practical and impractical are:
Magnesium: An expensive material. Castings are the only
readily available forms. Special precaution must be taken when
machining magnesium because this metal burns when hot.
Titanium: A very expensive material. Very tough and
difficult to machine.
Carbon Fibres: Still very expensive materials.
Kevlar Fibres: Very expensive and also critical to work
with because it is hard to "soak" in the resin. When this
technique is mastered, the resulting structure is very strong,
but it also lacks in stiffness.
The values given in our Table are for fibreglass with polyester
resins, which is very easy to use compared to the more critical
(viscous) epoxy fibreglass. Epoxy fibreglass provides a somewhat
stiffer and stronger result. ("Prepreg," epoxy pre-impregnated
cloth, is still very expensive, has a limited shelf life and
needs pressure as well as an oven to cure).
Aluminium Alloy 7075 - "T-whatever", has been left out
intentionally as it is a very strong but also very brittle
alloy. It is comparable to glass. Unless we state a "life" for a
specified part made of 7075, it is unsafe to use this alloy in
most light aircraft. (We are not an airline with an on-going
maintenance schedule - we want to fly our planes year after year
without having to worry about fatigue of our aircraft structure,
something we'll talk about later.)
Columns 2 through 6:
Columns 2 through 6 list the relevant material properties in
metric units. The multiplying factor on the bottom line will
transform the figures into North American Units.
Column 2, the density (d), is the weight divided by the volume.
Column 3, the yield stress (fy), is the stress (load per area)
at which there will be a permanent deformation after unloading
(the material has yielded, given way ... ).
Materials |
d |
fy |
fu |
e |
E/103 |
E/d |
Root2
of N/d |
Root3
of E/d |
fu/d |
|
1 |
2 |
3 |
4 |
5 |
6 |
7 |
8 |
9 |
10 |
Wood |
Spruce |
.45 |
- |
3.5/11 |
- |
1.4 |
2200 |
70 |
22.0 |
(15) |
|
Poplar |
.43 |
- |
30/12 |
- |
1.0 |
2200 |
70 |
22.0 |
(15) |
|
Oregon Pine |
.56 |
- |
4.0/13 |
- |
1.5 |
2200 |
70 |
22.0 |
(15) |
Fibreglass |
Matte |
2.2 |
- |
15 |
- |
1.5 |
700 |
17 |
5.0 |
7 |
(70% Glass) |
Woven |
2.2 |
- |
35 |
- |
2.0 |
900 |
20 |
6.0 |
16 |
|
Unidirectional |
2.2 |
- |
60 |
- |
3.5 |
1500 |
27 |
7.0 |
27 |
Alum. Alloy |
5052-H34 |
2.7 |
16 |
24 |
4 |
7.1 |
2600 |
30 |
7.0 |
11 |
|
8086-H34 |
2.7 |
22 |
31 |
5 |
7.1 |
2600 |
30 |
7.0 |
11 |
|
6061 -T6 |
2.7 |
24 |
26 |
9 |
7.1 |
2600 |
30 |
7.0 |
11 |
|
6351 -T6 |
2.7 |
25 |
28 |
9 |
7.1 |
2600 |
30 |
7.0 |
11 |
|
6063-T6 |
2.7 |
17 |
21 |
9 |
7.1 |
2600 |
30 |
7.0 |
11 |
|
2024-T3 |
2.8 |
25 |
41 |
12 |
7.2 |
2600 |
30 |
7.0 |
14 |
Steel AISI |
1026 |
7.8 |
25 |
38 |
15 |
21.0 |
2700 |
18 |
3.5 |
5 |
|
4130 N (4140) |
7.8 |
42 |
63 |
10 |
21.0 |
2700 |
18 |
3.5 |
7 |
Lead |
11.3 |
- |
- |
- |
- |
- |
- |
- |
- |
Magnesium Alloy |
1.8 |
20 |
30 |
- |
4.5 |
2500 |
37 |
9.0 |
16 |
Titanium |
4.5 |
50 |
80 |
- |
11.0 |
2400 |
23 |
5.0 |
18 |
|
Units for above |
kg/dm3 |
kg/mm2 |
kg/mm2 |
% |
kg/mm2 |
km |
kg-m2 |
kg2/3m1/3 |
km |
to obtain: |
lbs/cu3 |
KSI |
KSI |
% |
KSI |
|
|
|
|
multiply by: |
.0357 |
1420 |
1420 |
- |
1420 |
|
|
|
|
Note to
Table: The units used are the usual Metric S.I. (or MKFS)
international technical system where kg f = kg force (not mass
as in the Metric MKS, used in physics.) The usual North American
units and the conversion factors are also supplied in the bottom
lines.
Column 4, the ultimate stress (fu), is the stress (load per
area) at which it cannot carry a further load increase. It is
the maximum load before failure.
Column 5, the elongation at ultimate stress (e), in percentage
gives an indication of the 'Toughness" of the material.
Column 6 lists the Yongs Modular or Modulus of Elasticity (E),
which is the steepness of the stress/strain diagram as shown in
Figure 1.
Important Note: For wood, the tension is much greater (2 to 3
times) than the compression. Both values are given in the Table.
For fibreglass, the same applies, but the yield is so dependent
on the manufacturing process that we cannot even give 'typical
values'.
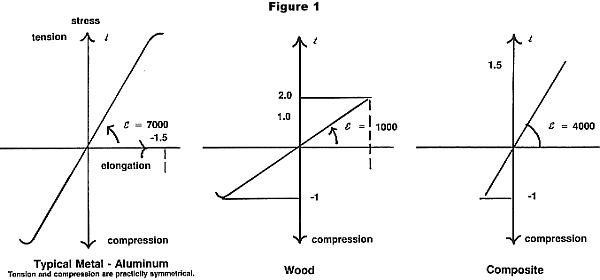
Both wood and
fibreglass need special analysis procedures to predict the
strength of a specific structural member. This analysis is quite
different from classic strength of material formulas. Today we
have to warn the "I wood' be designer" that his off-the-shelf
computer program may be okay for metal, but not for wood and
composites, even with the so-called "averaging" factors. We will
not discuss further here, but the serious student may want a
comprehensive textbook for engineers - not technicians who do
not have enough mathematical background. (STRENGTH OF MATERIALS
by Timoshenko, is a recommended sourcebook - Timoshenko,
STRENGTH OF MATERIALS, Part 1, 1955, "Elementary Theory and
Problems", $24.95; Part 11, 1956, "Advanced Theory and
Problems", $31.50. Available from Krueger Publishing Company,
P.O. Box 9542, Melbourne, FL 32902-9542.)
You see, math formulas and computers are tools like, say, a
planer. if you know how to set them, where and how to use them,
you can do very well with them. But if you play the sorcerer's
apprentice, it becomes dangerous for the tool, the operator and
the material.
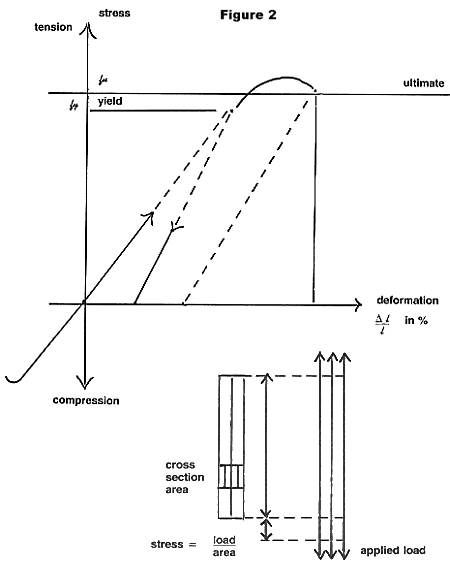
Columns 7 to 10:
Columns 7 to 10 are values which allow the comparison of
materials from a weight standpoint (the above referenced text by
Timoshenko will also show you why we use those "funny" looking
values).
Column 7 gives the stiffness of a sandwich construction. The
higher the value, the stiffer the construction. From the Table,
we see that metals are high wood comes close, but fibreglass is
low: which means fibreglass will be heavier for the same
stiffness.
Column 8 shows the column buckling resistance for the same
geometric shapes. This time, wood is better than the light
alloys, coming before steel and fibreglass. (Surprisingly, the
usual welded steel tube fuselage is not very weight efficient.)
Column 9 gives the plate buckling stiffness, which is also a
shear strength measure. Here again, wood (plywood) is in a very
good position before aluminium and fibreglass, with steel not
very good.
Column 10 provides a crude way of measuring the strength to
weight ratio of materials because it does not take into account
the various ways the material is used in "light structures".
According to this primitive way of looking, unidirectional
fibres are very good, followed by high strength (2024) aluminium
and wood, then the more common aluminium alloys and finally
steel.
From just this simple table, we find there is not one material
that provides an overwhelming solution to all the factors that
must be considered in designing a light aircraft. Each material
has some advantage somewhere. The designer's choice (no
preconceived idea) will make a good aircraft structure ... if
the choice is good!
|